In the strange and beautiful world of quantum physics, even stillness hides motion. Electrons whirl in orbits, wavefunctions ripple through space, and entire systems pulse with unseen rhythms. But what happens when physicists themselves take the reins of time—driving quantum systems with periodic pulses, shaking their very foundations in a precisely choreographed dance?
This is the idea behind periodic driving, also known as Floquet engineering. It’s a technique that introduces time-dependent changes to a system’s parameters, much like strumming a chord or setting a pendulum swinging. Unlike in classical systems, such rhythmic manipulation in quantum matter can unlock new, otherwise forbidden phases—realms of physics inaccessible in static conditions. These new phases are not theoretical curiosities: they hold profound implications for materials science, quantum computing, and our understanding of the fundamental nature of matter.
Now, in a groundbreaking experiment, researchers at Ludwig-Maximilians-Universität, the Max Planck Institute for Quantum Optics, and the Munich Center for Quantum Science and Technology have brought one such exotic phase into reality. By combining Floquet engineering with cutting-edge quantum simulation using ultracold atoms, they’ve created a large-scale, strongly interacting phase of matter known as the Mott-Meissner state—an elusive cousin of the famous quantum Hall states. Published in Nature Physics, their work paves the way for exploring the deep terrain of topological quantum phases and strongly correlated many-body systems.
Simulating Magnetism Without Magnets
At the heart of this achievement lies a powerful trick: creating synthetic gauge fields. Normally, electric and magnetic fields emerge from the movement of charged particles. But what if one could mimic their effects using neutral atoms—particles with no net charge—by engineering how they hop and interact in a lattice?
That’s exactly what Floquet engineering offers. By shaking an optical lattice—an artificial crystal made of light—scientists can force atoms to behave as though they’re experiencing a magnetic field, even though no real magnets are involved. The atoms, guided by cleverly tuned lasers, “feel” the curvature of synthetic space, curving their paths just as electrons do in a magnetic field.
This sleight of hand opens new frontiers. In natural solids, understanding strongly correlated phases—like those in high-temperature superconductors or fractional quantum Hall systems—is notoriously difficult. But with quantum simulators built from ultracold atoms, scientists can reconstruct such systems from the ground up, controlling every interaction and observing every motion.
Until now, however, a persistent obstacle has haunted these efforts: heating. Periodic driving, while essential for synthetic fields, tends to pump unwanted energy into the system. For non-interacting or weakly interacting atoms, this may be manageable. But for strongly interacting many-body systems, which are exquisitely fragile, heating can obliterate the very quantum states researchers hope to study.
Into the Ladder: Building the Mott-Meissner Phase
Enter Alexander Impertro and his colleagues. To overcome the heating problem and simulate robust many-body physics under synthetic magnetic fields, they designed a novel experimental platform based on bosonic flux ladders—a special kind of low-dimensional system resembling a ladder where atoms can hop along the legs and across the rungs.
Using a two-dimensional optical lattice, they partitioned space into an array of independent ladder systems. Each ladder was formed from optical superlattices—patterns of light with variable spacing that allowed precise control over how atoms moved and interacted. On each rung of the ladder, they applied Floquet engineering to imprint the effects of a magnetic field. The atoms, caught in this luminous web, began to behave as if under the influence of a synthetic magnetic flux.
But imposing a field was only half the story. The real challenge was introducing strong interactions—making the atoms not just behave independently, but talk to each other, push against one another, and collectively form new quantum states.
To achieve this, the team used a key property of cesium atoms known as a Feshbach resonance. This phenomenon allows scientists to dial up or down the strength of interactions between atoms by tuning an external magnetic field. Like adjusting the tension on guitar strings, the researchers could now fine-tune their quantum material until it played the desired tune.
What emerged from this intricate setup was a robust realization of the Mott-Meissner phase, a peculiar state of matter where quantum particles flow persistently without dissipation, forming patterns of circulating currents even in the absence of external forces.
Seeing the Invisible: Measuring Quantum Currents
But how do you know what your quantum material is doing? You can’t touch it. You can’t see it. Even traditional tools like quantum gas microscopes fall short: they measure local densities—how many atoms are in each site—but cannot detect currents, the invisible rivers of motion that define phases like the Mott-Meissner state.
So the team devised an ingenious solution. Building on earlier work, they implemented a current detection technique adapted for use in their Floquet-engineered ladders. With this new tool, they could observe, with full spatial resolution, how atoms were flowing—where they formed loops, how strong the currents were, and how the pattern changed with varying interaction strength.
For the first time, a large-scale, low-temperature, strongly interacting Floquet system could be studied not just in theory or simulation, but in real laboratory conditions. The team mapped out the persistent currents in the Mott-Meissner phase and compared them with predictions from numerical simulations, confirming that the system had indeed cooled into the desired quantum state.
Even more remarkably, by matching the experimental data to theory, they could estimate the effective temperature of their system—a key parameter for exploring more delicate phases in the future.
Beyond the Horizon: Towards Fractional Quantum Hall Physics
The implications of this achievement are far-reaching. Many of the most tantalizing states in modern physics—fractional quantum Hall states, anyonic excitations, and topological order—exist in a landscape beyond traditional solids. Simulating them requires not just synthetic gauge fields, but strong interactions, low temperatures, and precision measurements.
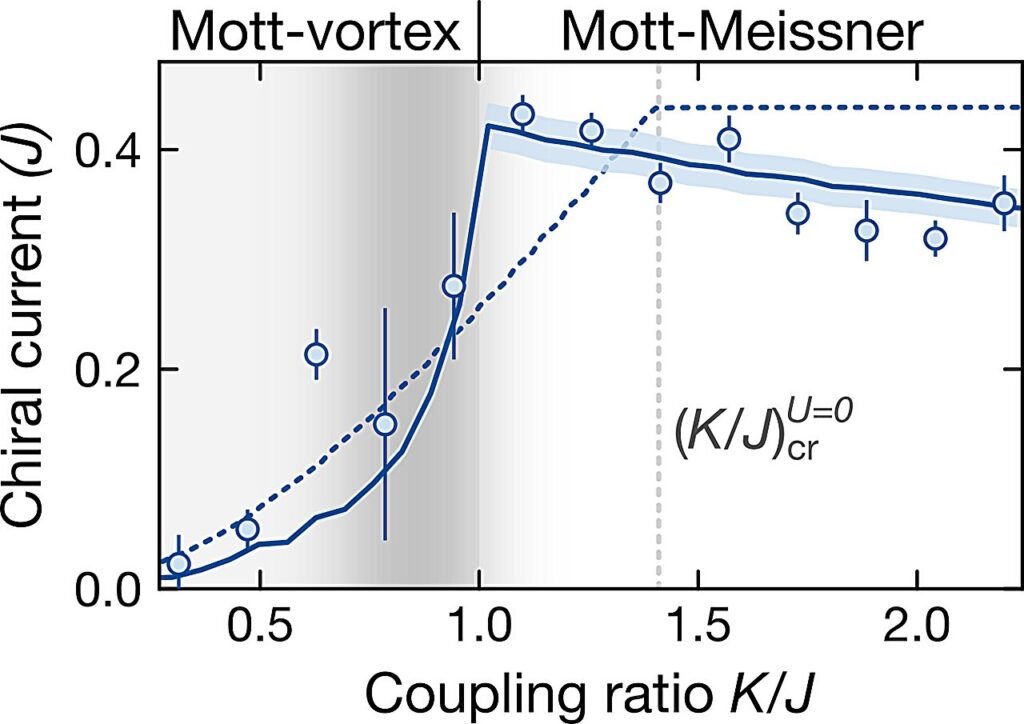
Impertro and his colleagues have now shown that this is possible. With their platform, they’ve cracked the barrier that previously limited such studies to systems with only one or two particles. Their ladders contain dozens of interacting particles, all governed by synthetic magnetism and observed with microscopic clarity.
This opens a new playground for quantum simulation. Not just for understanding exotic physics, but for testing quantum materials that might one day form the backbone of fault-tolerant quantum computers or next-generation electronics. Systems that obey non-Abelian statistics or harbor Majorana zero modes may one day be built from the same foundational ideas demonstrated here.
The Road Ahead: Complexity, Fragility, and the Quantum Frontier
The team is far from done. Their next steps involve exploring the full phase diagram of interacting flux ladders. While the Mott-Meissner state marks a significant milestone, many more exotic states lie hidden in the map—vortex phases, symmetry-broken states, and possibly even fractionalized excitations reminiscent of those in topological insulators.
To access these, they’ll need to reduce temperatures even further and refine their control over the system’s parameters. They also aim to extend from ladders to full two-dimensional systems, where the most exotic phases of matter—like the fractional quantum Hall effect with anyonic particles—reside.
Such progress won’t come easily. Every additional degree of freedom introduces complexity. Every attempt to go colder risks failure. But the roadmap is now clearer, and the tools more powerful.
Conclusion: Taming Time to Rewrite Matter
In their quest to simulate the unseeable, Impertro and his colleagues have shown how time itself can be sculpted—not just as a backdrop for motion, but as an active tool for shaping the quantum world. Floquet engineering is no longer just a theoretical toy. With sufficient care, it becomes a portal into new quantum phases once thought unreachable.
Their experiment isn’t just a technical triumph; it’s a philosophical one. It challenges the assumption that real magnetism, real materials, or even real charges are necessary to explore nature’s deepest laws. In the laboratory of light and time, reality becomes programmable.
And in that programmable reality, where atoms dance to invisible rhythms and fields are born from laser pulses, we find ourselves at the dawn of a new age—not just of understanding matter, but of creating it.
Reference: Alexander Impertro et al, Strongly interacting Meissner phases in large bosonic flux ladders, Nature Physics (2025). DOI: 10.1038/s41567-025-02890-0. On arXiv: DOI: 10.48550/arxiv.2412.09481