In the world of optics, there’s a well-known challenge that has puzzled scientists and engineers alike: how do you focus light into a space smaller than its own wavelength? It’s a bit like trying to squeeze a car into a matchbox. Light, after all, has physical limits—its wavelength defines the smallest area it usually occupies. But for photonic technology to reach its full potential, from ultra-compact sensors to next-gen quantum computers, we need to beat that limit.
Now, researchers from AMOLF in the Netherlands, TU Delft, and Cornell University in the United States have done just that. They’ve discovered a completely new way to focus and amplify light into an incredibly tiny volume—one no bigger than the light’s own wavelength. Their work, published in Science Advances on April 18, could become a cornerstone of future photonic chips and quantum communication systems. What makes their breakthrough even more exciting is that it doesn’t just work for one color or type of light—it works across a broad spectrum, overcoming one of the major hurdles of previous methods.
Why We Need to Focus Light So Precisely
At first glance, focusing light might seem like an ordinary task. After all, we do it all the time with magnifying glasses and lenses. But on the microscopic scale of photonic chips, where devices are only a few hundred nanometers wide (a thousand times thinner than a human hair), traditional lenses don’t cut it. You can’t just stick a glass lens onto a silicon chip and expect it to work.
Concentrating light at this scale is crucial for several modern technologies. It allows for more precise control in quantum communication, enhances optical sensors used in biological and chemical detection, and can improve the efficiency of on-chip lasers that serve as light sources in photonic circuits. Until now, there were two main strategies to focus light: using optical cavities or waveguides.
Optical cavities trap light in a small region through resonance—like sound echoing inside a chamber. The problem is, this only works at very specific wavelengths, limiting the range of applications. The second method, waveguide tapering, acts like a funnel that squeezes light down to a point. This method is broader in application but still limited by diffraction and geometry.
“We needed a method that didn’t just squeeze light into a tight spot, but did it across multiple wavelengths and without significant loss,” explains Ewold Verhagen, group leader at AMOLF. That’s where the new idea comes in—one that plays with the fundamental rules of topology, not just optics.
The Topological Twist
The new approach is rooted in something called topological photonics, a cutting-edge field inspired by concepts from mathematics and quantum physics. In simple terms, topology is the study of properties that remain unchanged when an object is stretched or twisted—but not torn. Think of a doughnut and a coffee cup; topologically, they’re the same because each has one hole.
Now imagine applying those mathematical rules to light. In a physical system like a photonic crystal—a silicon slab with a regular pattern of holes—these topological principles can control how light moves through the material.
Here’s how it works: when two specially designed photonic crystals are placed next to each other with mirrored hole patterns, they create a boundary along which light is forced to travel. This boundary acts like a custom-made highway for photons, but with a twist: the movement of light along it is topologically protected. That means it can’t be easily stopped or reflected by imperfections or tiny obstacles—something that usually causes major issues in nanoscale optics.
“We created a kind of ‘light trap’ where photons travel safely along this waveguide without scattering,” explains Daniel Muis, the Ph.D. student who led the experimental effort.
The Light Hits a Wall—and Amplifies
But what happens if this light highway suddenly ends in a dead-end? A wall of material through which light cannot pass?
This is exactly what the team explored. Based on theoretical predictions by Gennady Shvets and colleagues at Cornell University, they expected that when the waveguide abruptly ends, something surprising would happen. Instead of bouncing back immediately, the light would accumulate at the boundary. It would gather, concentrate, and linger—resulting in an amplified light field.
This is possible because the usual reflection that occurs when light hits a barrier is strongly suppressed due to the system’s topology. Essentially, the light doesn’t know how to escape easily. It hesitates, it waits—and during that delay, the light intensity at the edge builds up to levels that would be impossible using conventional methods.
This new form of light concentration doesn’t rely on the precise tuning of wavelength or cavity size. It works for a wide range of wavelengths, offering a broadband solution for one of the thorniest problems in photonics.
Visualizing the Invisible
To verify this behavior, the team had to literally “see” the accumulation of light on the chip. That’s no easy feat, considering the resolution needed is at the nanometer scale. At TU Delft, Muis used a specialized microscope that can detect optical fields using an ultra-fine needle hovering just above the surface of the chip—like a nanoscale antenna.
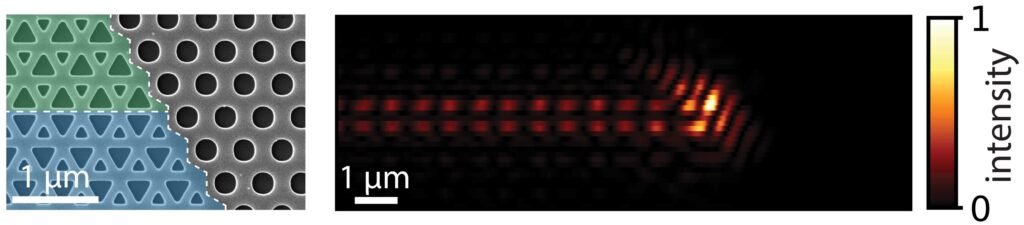
The results were stunning. “We saw a very clear amplification of the light field precisely at the location we expected,” says Muis. Even more impressive was the discovery that this effect only occurred when the terminating wall was placed at a specific angle—exactly as predicted by the theory. This angular sensitivity further confirmed the topological nature of the phenomenon.
A Universal Blueprint for Wave Control
The implications of this discovery stretch far beyond just photonic chips. Because the behavior arises from the structure of the medium—not the material or even the type of wave—the principle can be applied to other systems. That includes acoustic waves (sound), mechanical vibrations, and even electron waves in certain crystalline solids.
In other words, this is not just a new way to concentrate light—it’s a new framework for controlling all kinds of waves in structured media.
The researchers believe the method could lead to improved designs for photonic devices, especially in areas where multiple wavelengths need to be used simultaneously, such as biomedical imaging, optical computing, and secure quantum data transfer.
Next Frontiers: Pushing the Boundaries Further
What’s next for this team of light sculptors?
Muis and his colleagues are already planning to take the experiment further. One idea is to use pulsed lasers instead of continuous beams, allowing them to study the exact time scale over which light accumulates at the boundary. That could reveal just how far the amplification can go—and how fast it builds up.
Such insights could lead to devices that store and release energy in tiny bursts, or chips that manipulate light in previously unimaginable ways. Another goal is to integrate these topological structures into real-world photonic circuits, merging them with existing technology to make ultra-compact, high-speed optical processors.
“If we understand the time dynamics of the light accumulation, we could create new types of delay lines, amplifiers, or even novel lasers that operate at the limits of physical confinement,” says Muis.
A New Era in Light Control
This breakthrough represents a turning point in our ability to control light. By leveraging the strange but powerful rules of topology, researchers have achieved what was once thought impossible: confining and amplifying light within a volume as small as the light’s own wavelength—without sacrificing bandwidth or flexibility.
It’s a triumph not just of physics, but of interdisciplinary collaboration. From theoretical modeling at Cornell to nanofabrication at AMOLF and real-time visualization at TU Delft, the research blends mathematics, engineering, quantum physics, and material science.
The next time you use a sensor, stream quantum-secured data, or benefit from more efficient photonic chips, the technology behind it might be rooted in this very discovery: light that doesn’t bounce, doesn’t scatter—just waits, builds, and blazes in a confined space.
Reference: Daniel Muis et al, Broadband localization of light at the termination of a topological photonic waveguide, Science Advances (2025). DOI: 10.1126/sciadv.adr9569. www.science.org/doi/10.1126/sciadv.adr9569